
Society based on hydrogen: present and future
The development of renewable energy sources and the increasing demand for energy resources have led to numerous issues in energy and the environment. Successfully constructing a hydrogen society requires technological advancements and rational design of hydrogen production, storage, delivery, and usage. A recent paper published in “Energy & Environmental Science” provides systematic insights into recent attainments, limitations, and future directions of hydrogen production, storage, delivery, and usage combined with some original techno-economic calculations.
The authors argue that hydrogen should play a critical and indispensable role in future long-term energy systems due to its high calorific value, zero-carbon feature, and environmental-friendly merit. The proportion of hydrogen energy in the total final energy consumption should increase to 2% and 10% in 2030 and 2050, respectively, to realize the net-zero plan.
The global hydrogen demand has increased significantly, with projections of increased demand in 2030 and 2050. Hydrogen is primarily used in refining, ammonia synthesis, and industrial processes but is expected to be increasingly used in transport, power, NH3 fuel, synfuels, buildings, and grid injection. By 2050, hydrogen-value chains will require $7-8 trillion investment, creating $3 trillion in revenues through the hydrogen economy. Zero-carbon hydrogen is crucial for CO2 emission reduction, and the IEA recommends a 4.3 trillion USD investment in 2030.
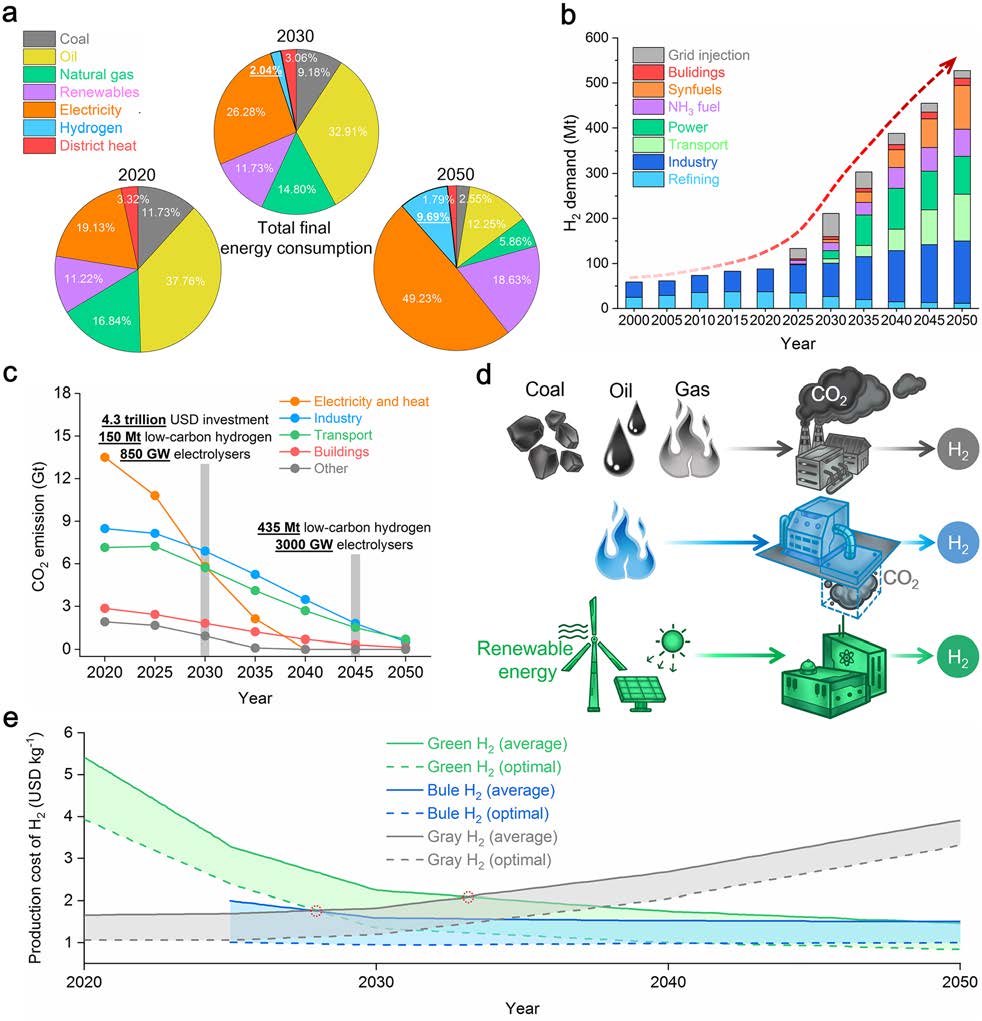
Hydrogen can be divided into grey, blue, and green hydrogen modes. Grey hydrogen accounts for 95% of all produced hydrogen resources, while blue hydrogen comes from steam methane reforming or gasification using carbon capture and storage technologies. Green hydrogen is expected to replace grey hydrogen in 2030.
Green hydrogen production can be achieved through various renewable resources, including electrolysis, photocatalysis, solar thermochemical water-splitting cycle, photovoltaic electrolysis, supercritical water gasification of biomass, and combined dark fermentation and anaerobic digestion. Electrochemical water splitting is relatively mature and beneficial for large-scale hydrogen production.
Five typical water electrolysis technologies include alkaline water electrolyser (AWE), anion exchange membrane electrolyser (AEME), proton exchange membrane electrolyser (PEME), solid oxide electrolysis cell (SOEC), and prototonic ceramic electrolysis cell (PCEC). However, AWE technology faces challenges such as slow start times, inability to quickly start or shut down, and potential insoluble KOH compounds.
AEME, a solid electrolyte, was developed to overcome the shortcomings of AWE, achieving larger current densities, faster cold start to nominal load, and higher voltage efficiency than AWE at the laboratory scale. However, recent laboratory-scale AEME membranes show poor chemical and mechanical stability and low conductivity, resulting in a poor lifetime. PEME, a solid electrolyte/separator, is chemically and mechanically stable, enabling effective transport of H+ protons and offering high cell pressure, wide load range, and long lifetime. However, PEME faces challenges such as fouling and expensive noble metals. Proton-conducting PCEC technology, based on perovskite oxides, is a promising next-generation technology for hydrogen production.
Renewable solar energy can be used for water splitting, with photocatalysis being cost-effective and simple. Photocatalysis can split water into H2 and O2, but its efficiency is limited to 1%. Recent research has improved the efficiency of photocatalysis by 9.2%, but further improvements are needed to achieve commercial cost targets. The solar thermochemical water-splitting cycle can transform solar energy into thermal energy via solar collectors. However, the efficiency and stability of photocatalysis need to be enhanced for commercialization.
The cost of green hydrogen production mainly comes from electricity consumption. Designing efficient and stable electrocatalysts to reduce electricity consumption is crucial for optimizing green hydrogen technologies.
Water electrolysers for green hydrogen production use HER and OER electrocatalysts, which can operate in acidic, alkaline, neutral, seawater, or solid-state electrolytes. Current state-of-the-art catalysts are expensive and scarce, with promising candidates like noble metal-based Pt and nonprecious materials. Alkaline water electrolysis has enhanced stabilities, but alkaline HER is slower due to water interactions. Neutral water splitting can address issues like corrosive working environments and expensive cation/anion exchange membranes. High-temperature solid-state SOEC and PCEC are more efficient, but stability levels need to be increased.
Nanotechnologies are being used to increase material surface areas and improve catalysis performance. By reducing catalyst particle size, they can increase active surface areas and expose materials to catalysis reactions. This is known as the size effect. The engineering of nanomaterials has developed rapidly, from 3D nanoparticles to 0D single atoms. Introducing multiple elements into electrocatalysts can enhance catalytic efficiency. Optimizing material properties, such as contact angle, magnetism, and electron transfer capability, can improve catalytic efficiency and water electrolysis performance.
Material structural properties play a crucial role in the activity and stability of water electrolysis. The smallest structural units, such as tetrahedral, pyramidal, and octahedral, can exert different coordination chemical effects to adsorb/desorb reactants, intermediates, and products, affecting the catalysis performance. Understanding material molecular-level structures is important for rational catalyst design, as material structures induce strain, and a strain-tuning strategy effectively optimizes water electrolysis performance. Under electrochemical water electrolysis conditions, oxidation and reduction processes are triggered by variations in material electronic structural properties, including valence, spin, and orbital states. Material eg occupancy, covalence, band center, charge transfer energy, and valence state, can be successfully exploited as activity descriptors to guide material design for water electrolysis. To effectively screen and exploit active and robust electrocatalysts for green hydrogen production, rational and instrumental pathways and methodologies are shown.
The full article can be found here: https://doi.org/10.1039/D3EE02695G
Taking the forefront in the movement towards a sustainable energy future through the implementation of eco-friendly ammonia and hydrogen solutions